The disease we want to fight:
Lysosomal Storage Disorders (LSDs)
Lysosomal storage diseases (LSDs) comprise more than 70 inherited metabolic disorders characterized by the disruption of the normal lysosomal function due to defects on lysosomal proteins. Although individually these are rare or extremely
rare disorders, as a group the incidence of LSDs is estimated in 1 out of 5,000 live births
1, posing a heavy human and economic burden on the whole society
2–4.
In LSDs the loss of the enzymatic or transporter activities leads to accumulation of unprocessed metabolites within the lysosomal compartment of the cells, which can be toxic to cells and result in multiple organ damage
5. This malfunctioning translates in a spectrum of clinical symptoms (different depending on the specific disease). Importantly, two-thirds of LSD patients manifest severe neurological symptoms and many die early in life.
Some examples of LSDs include: Gaucher disease, Fabry disease, Pompe disease, mucopolysaccharidosis, metachromatic leukodystrophy, pycnodysostosis, cystinosis, Niemann-Pick disease or acid lipase deficiency.
LSDs and Enzyme Replacement Therapy (ERT)
LSDs are considered orphan diseases due to their low incidence and very limited therapeutic options. Among these options we find specific enzyme replacement therapy (ERT) with recombinant human enzymes or a handful of supportive measures 6–8. ERT is usually the preferred option, but it comes with several drawbacks limit its application and effectiveness:
1- Poor biodistribution of the enzyme: Leading to insufficient reversal of the pathology in tissues such as muscle, bone, cartilage, heart and lung 9
2- Enzymes instability: Low plasma half-life of recombinant enzymes forces repetitive intravenous (i.v.) administration of high enzyme doses 10
3- Generation of autoantibodies: Antibodies against the recombinant enzyme may reduce its efficacy requiring the administration of increasingly higher doses to maintain the therapeutic effect.
4- Inability of enzymes to cross the blood-brain barrier: Enzymes cannot reach the brain upon intravenous administration and alternative methods to deliver the enzyme to the brain tend to be highly invasive 11.
5- Ineffectiveness for LSD caused by non-enzymatic proteins: Due to the technical difficulty of recombinantly producing transmembrane proteins ERT is only amenable to LSDs whose deleterious lysosomal protein is an enzyme.
Nanotechnology and the use of drug delivery systems has been proposed as an ideal approach to overcome these problems 12.
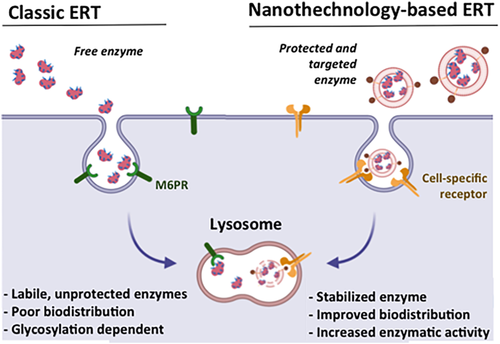
Fig. 1: Comparison of the classical enzyme replacement therapy (classic ERT) vs. nanotechnology-based ERT. ERT relays on the intravenous administration of very labile lysosomal enzymes that require complex glycosylation patterns to internalize into cells. Nanotechnology-based approaches allow improving ERT by stabilizing the enzyme, improving its biodistribution and delivering the therapeutic protein directly to the lysosomes of affected cells. From 12.
Extracellular Vesicles (EVs) for LSDs and other applications
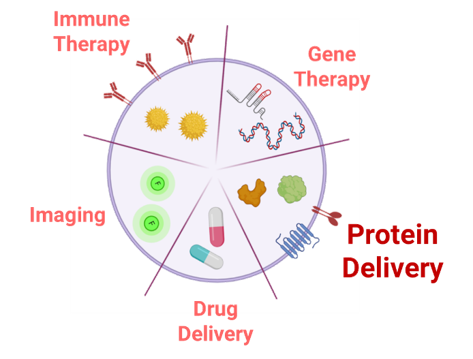
Fig. 2: Representative scheme of potential uses as delivery systems for EVs in the biomedical context.
Extracellular Vesicles (EVs), are heterogenous cell secreted membrane bound particles crucial in cell-to-cell communication. Particularly, exosomes (30-100 nm) and microvesicles (100-1000 nm) are the EVs types that have raised a higher interest as natural signaling nano-platforms 13–15. These EVs have been shown to shuttle a large number of macromolecules, including proteins, nucleic acid, lipids and other metabolites to various tissues and organs 16,17. Interestingly, EVs’ high biocompatibility, low immunogenicity and intrinsic tissue-penetrating ability , make them optimal nano-carriers for biomedical applications (Figure 1) over other existing drug delivery systems (DDS) such as liposomes, polymeric nanoparticles and gold nanoparticles 18–20. Additionally, delivery of proteins in conventional nanotechnology-based carriers, require tedious protein production and purification steps and the amount of protein loaded with this kind of procedures is often poor 20. Conversely, protein over expression in EV-secreting cells combines protein production and nanoparticle loading in a single step. In the LSD landscape, it is worth mentioning that EVs, which have been described to enter the cells mainly through endocytic processes 22, naturally lead the EVs cargo towards the final targeted subcellular compartment, the lysosome, where the therapeutic action is required.
Moreover, EVs are also especially suited as vehicles for membrane proteins, since their natural insertion to EVs membrane, prevent protein aggregation during purification 24. Indeed, a significant fraction of EVs’ proteome corresponds to membrane associated proteins 24.
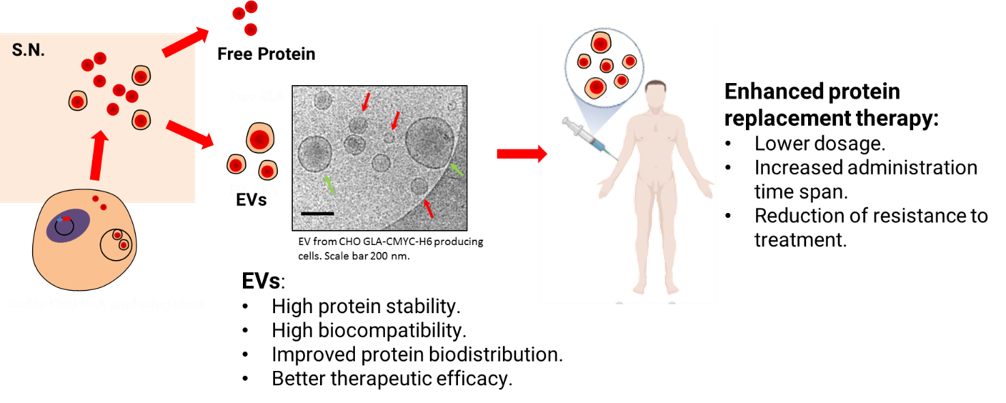
Fig. 3: Representative scheme showing the production and loading of therapeutic proteins by recombinant protein overexpression in mammalian cell factories and their prospected use as enhanced protein replacement therapies.
Both liposomes and natural extracellular vesicles (EVs) have gained growing therapeutic interest in recent years due to their properties, which make them extremely suitable for nanomedicine research and applications. EVs have been extensively studied as possible nanocarriers to exploit their natural biological role for therapeutic purposes. However, there are still some potential drawbacks and unknown aspects that can limit their medical applicability, such as difficulties in EV extraction (with particular focus on the process yield), characterization, mass production and their rapid clearance from the blood when EVs are systemically administered. To overcome these difficulties, different approaches have been explored so far, starting from the extracellular vesicle surface modification to obtain engineered EVs, up to the development of hybrid EVs achieved through the fusion of their membrane with liposomes to combine the unique features that these two organic structures provide.
The MIMIC-KeY approach
In MIMIC-Key project we work on the development of artificial EVs, characterized by a lipidic bilayer composed of artificial lipids enriched with specific proteins in order to mimic the biological features
of natural extracellular vesicles, like homing capabilities to target cells and ability to transfer a therapeutic cargo. Furthermore, we wish to address this challenging task by encapsulating stimuli-responsive breakable porous nanoparticles
within the engineered lipidic shell. This approach is at the heart of nanomedicine research, especially as effective drug delivery systems, here customized to deliver the therapeutic enzyme to fight the pycnodisostosis disease. Such
fully artificial EVs are conceived to be artificially built in a bottom-to-up approach, starting from well-characterized biomolecules (principally phospholipids and proteins) and employing only the key elements,
to obtain an EV-resembling nanotool, with the desired functionalities. What is notable is that this strategy allows the possibility to bypass some of the obstacles related to natural EVs, such as the difficulty of EV extraction and
purification methods and the lack of standardized procedures; moreover, it paves the way for a more controlled, safer product with a higher pharmaceutical acceptability.
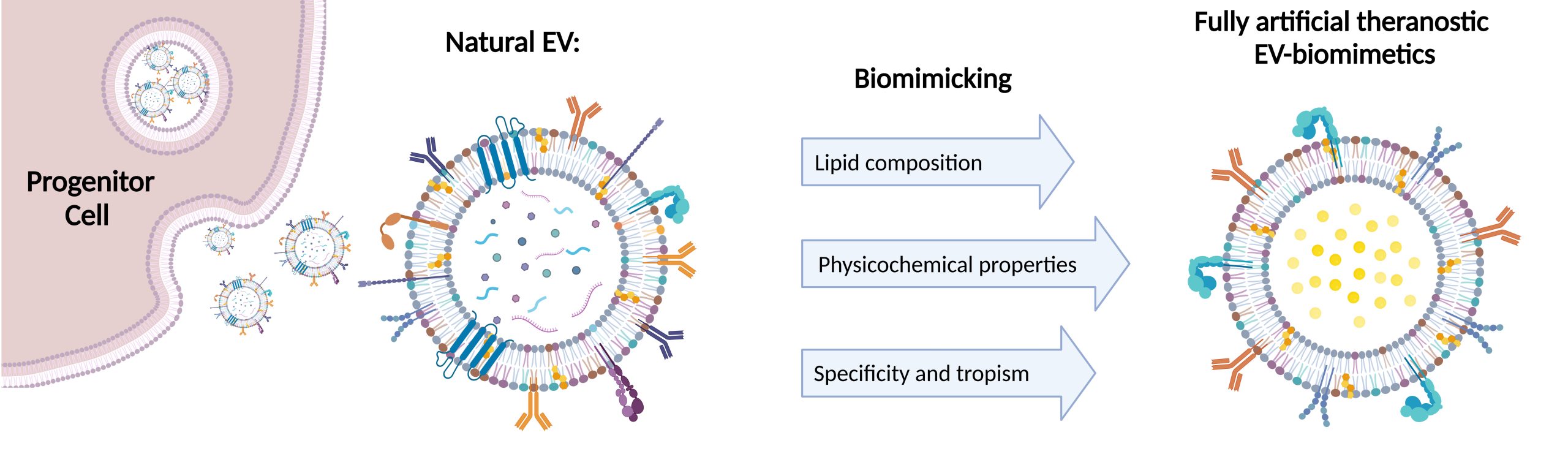
References
1 Platt, F. M. et al. Nat. Rev. Dis. Prim. 4, 27 (2018)
2 Parenti, G. et al. Annu. Rev. Med. 66, 471–486 (2015)
3 Futerman, A. H. et al. Nat. Rev. Mol. Cell Biol. 5, 554–65 (2004)
4 Beck, M. Human Genetics 121, 1–22 (2007)
5 Ballabio, A. et al. Biochimica et Biophysica Acta – Molecular Cell Research 1793, 684–696 (2009)
6 Lachmann, R. H. Current Opinion in Pediatrics 23, 588–593 (2011)
7 Wraith, J. E. J. Inherit. Metab. Dis. 29, 442–447 (2006)
8 Wyatt, K. et al. Health Technol. Assess. (Rockv). 16, 1–566 (2012)
9 Brady, R. O. Annu. Rev. Med. 57, 283–296 (2006)
10 Safary, A. et al. Bioimpacts 8, 153–157 (2018)
11 Bellettato, C. M. et al. ItalianJournal of Pediatrics 44, 131 (2018)
12. Abasolo, I. et al. WIRES Nanomed Nanobiotechnol. 13, e1684 (2021).
13 Mittelbrunn, M. et al. Nature Reviews Molecular Cell Biology 13, 328–335 (2012)
14 Record, M. et al. Biochimica et Biophysica Acta – Molecular and Cell Biology of Lipids 1841, 108–120 (2014)
15 Raposo, G. et al. Journal of Cell Biology 200, 373–383 (2013)
16 Choi, D. S. et al. Proteomics 13, 1554–1571 (2013)
17 El Andaloussi, S. et al. Nature Reviews Drug Discovery 12, 347–357 (2013)
18 Kumari, A. et al. Colloids and Surfaces B: Biointerfaces 75, 1–18 (2010)
19 Sahay, G. et al. Nat. Biotechnol. 31, 653–658 (2013)
20 Haney, M. J. et al. J. Control. Release (2015)
21 Yu, M. et al. J. Control. Release 240, 24–37 (2016)
22 Rufino-Ramos, D. et al. (2017)
23 Seras-Franzoso, J. et al. J. Extracell. vesicles (2021)
24 Yang, Y. et al. Journal of Extracellular Vesicles 7, (2018)